An
understanding of the morphology,
physiology, and electrophysiology of
peripheral nerve regeneration is
fundamental to clinical
decision-making by the peripheral
nerve surgeon. Questions regarding
the type and timing of nerve repair
can be addressed logically only if
the underlying condition of the
injured nerve can be ascertained.
After nerve injury, a detailed
history of the mechanism of injury
along with a directed physical
examination and electrophysiologic
studies are obtained by the surgeon.
The clinical course is followed
carefully in terms of improvement,
stabilization, or deterioration.
These data should then be
transformed into mental images of
the histology and physiology within
the nerve fascicles at the injury
site, and in the nerve segments
proximal and distal to that site.
Appropriate decisions can then be
made regarding the clinical options
of further observation, exploration,
and neurolysis with or without nerve
grafting, or reconstructive
procedures such as tendon transfers.
The morphology, physiology, and
electrophysiology of peripheral
nerve regeneration cannot be
analyzed in isolation. These
regenerative processes are a
continuum of the entire response to
nerve injury, including concomitant
degenerative processes.
Just as degenerative and
regenerative processes are
simultaneous and interdependent, so
are the structural and physiological
aspects of the responses to nerve
injury. They cannot be discussed in
isolation, either.
The
clinically relevant fundamentals of
the anatomic, physiologic, and
electrophysiologic changes observed
in injured and regenerating nerves
are reviewed. The discussion is
broad enough to apply to most major
mechanisms of nerve injury-traction
or stretch, contusion, laceration,
missile injury, injection,
entrapment, and compression.
Classification of Nerve Injuries
Figure 1 reviews briefly the major
categories of nerve injury. The two
most widely used classification
schemes are those of Seddon and
Sunderland. Seddon described three
basic types of nerve injury:
neurapraxia, axonotmesis. and
neurotmesis. These categories are
encompassed within Sunderland's
expanded classification: Neurapraxia
(conduction block) is equivalent to
Sunderland's first-degree injury;
axonotmesis is synonymous with
Sunderland's second-degree injury;
and neurotmesis is Sunderland's
fifth-degree or most severe injury.
All of these injuries initially
produce complete loss of neurologic
function. The clinical importance
for the peripheral nerve surgeon of
classification schemes lies in its
predictive value i.e.. its
prognostic significance.
First-Degree Injury (Neurapraxia)
In
terms of nerve regeneration.
first-degree injuries are not
strictly relevant in that no true
regeneration occurs. A first-degree
injury involves interruption of
signal conduction across the injured
site, usually due to compression or
ischemia, with full and relatively
rapid recovery of function. The axon
is preserved. there is no Wallerian
degeneration, and pathological
changes are mild and fully
reversible.
For unknown reasons, motor fibers
are more susceptible to this type of
injury than are sensory or
sympathetic fibers. Susceptibility
by modality, in descending order,
is: motor, proprioception, light
touch, temperature sensation, pain
sensation, and sympathetic function.
Recovery generally occurs in a
reverse sequence, and is usually
complete within days to a few
months. Any residual deficit
indicates a more severe degree of
injury involving loss of axonal
continuity.
Second-Degree Injury (Axonotmesis)
second-degree injury is defined
pathologically by loss of axonal
continuity and subsequent Wallerian
degeneration. The endoneurial sheath
is preserved, however, and each
regenerating axon is confined to its
original sheath. This ensures
faithful reinnervation of the
appropriate end organ, and full
functional recovery inevitably
results. In clinical terms, the
initial deficit involves complete
motor, sensory, and sympathetic
function. Nerve conduction distal to
the injury site disappears within
24-72 hours after the injury, and
fibrillation potentials (see
"Electrophysiology") are present
within the denervated muscles.
Recovery of motor function in
second-degree injury follows
sequentially from proximal to distal
muscles. The timing of recovery
follows quite predictably the rate
of axonal growth-approximately 1 mm
per day or 1 inch per month,
although the growth rate is more
complex when carefully studied as
described in the next section. The
progression of regeneration can be
followed along sensory fibers by
tracing the progress of Tinel's
sign. The delay in recovery of
function exceeds that observed after
first-degree injury, and is usually
measured in months rather than days
or weeks.
Third-Degree Injury
Third-degree injuries are
intrafascicular injuries that
involve disruption of axons as well
as their endoneurial tubes (basal
lamina of Schwann cells), with
subsequent Wallerian degeneration.
The perineurium is spared and,
therefore, the fascicular
architecture of the nerve is
preserved. Retrograde degeneration
is severe, and some neuronal cell
bodies are lost. reducing the number
of axons available for regeneration.
|
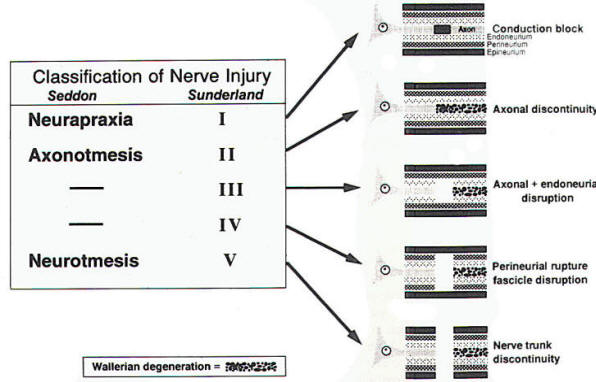 |
Fig-1:
Classification of nerve
injury by Seddon and
Sunderland |
Intrafascicular fibrosis (scar),
which results from the associated
hemorrhage, edema, and ischemia,
presents an impediment to axonal
regeneration. Recovery is,
therefore, incomplete. Regenerating
axons are confined within their
original fasciculi, but are no
longer confined within their
original endoneurial tubes.
Therefore, misdirected regrowth can
occur- e.g., sensory axons may
regenerate along original motor
tubes. This constitutes wasteful
regeneration. More proximal injuries
are more likely to result in
neuronal cell death and in a
significant proportion of
misdirected axons.
Recovery after third-degree injury
is considerably delayed. The Tinel's
sign is not a reliable marker of
functional recovery after
third-degree injury because sensory
axons may be descending along a
"dead-end" motor endoneurial tube.
The external appearance of such a
nerve will not accurately reflect
the severe degree of intrafascicular
disruption and disorganization
present-an important point for the
peripheral nerve surgeon to
remember.
Fourth-Degree Injury
This severe injury involves rupture
of the perineurium and, thus,
disrupted fasciculi. The nerve trunk
is still in continuity, but is
converted at the injury site into a
solid scar containing Schwann cells
and regenerating axons, which
enlarge to form a neuroma.
Retrograde neuronal effects are more
severe than in third-degree
injuries; therefore, even fewer
axons survive to regenerate. Those
axons that do regenerate are no
longer confined within fascicles,
and many stray into surrounding
interfascicular tissue to end
blindly. Few axons reach their
appropriate targets. Functional
recovery, if any, is usually quite
limited. Fourthdegree injuries
require surgical excision of the
involved segment and an appropriate
type of nerve repair.
Fifth-Degree Injury (Neurotmesis)
This most severe degree of nerve
injury involves complete loss of
nerve trunk continuity. The severed
nerve ends may remain separated, or
they may be joined by a scar-tissue
bridge composed of fibroblasts,
Schwann cells, and regenerating
axons. The extent of scar tissue
varies, but often there is a
proximal neuroma and distal bulb
that form either a dumbbell-shaped
structure or an amorphous mass of
scar tissue. Regeneration across
this formidable barrier is severely
limited, at best, and is
functionally negligible. Even with
resection and nerve repair,
significant barriers to full
recovery remain. These include loss
of axons due to retrograde effects
of the injury, and misdirected
axons. The chances of useful
recovery are markedly enhanced by an
appropriate surgical repair.
Morphologic and Physiologic Changes
after Nerve Injury/Repair
Degenerative Changes
Before regeneration of nerve fibers
can occur, a series of degenerative
processes must take place. Many of
these are direct preludes to
regeneration. The success of
regeneration depends to a large
extent upon the severity of the
initial injury and the resultant
degenerative changes. Pathologic
changes are mild or absent in
first-degree injuries in which
conduction block alone occurs, and
no true degeneration or regeneration
occurs.
In
second-degree injuries
(axonotmesis), there is little
histologic change at the site of the
injury or proximal to it. The major
changes occur distal to the injured
segment in what is well-known as
Wallerian, or anterograde
degeneration.
The primary histologic change in
this process involves physical
fragmentation of both axons and
myelin, which begins to appear
within hours of the injury.
Ultrastructurally, both neurotubules
and neurofilaments become
disarrayed, and axonal contour
becomes irregular, due to varicose
swellings. By 48-96 hours
postinjury, axonal continuity is
lost and conduction of impulses is
no longer possible. Myelin
disintegration lags slightly behind
that of axons, but is well advanced
by 36-48 hours.
Axonal and myelin debris is removed
by the phagocytic action of
macrophages and Schwann cells, a
process which can take from
1
week to several months. Schwann
cells become active within 24 hours
of the injury, exhibiting nuclear
and cytoplasmic enlargement as well
as an increased mitotic rate.
Schwann cells appear to ingest
axonal and myelin debris, and then
pass this on to macrophages. The
latter migrate into the traumatized
region, primarily through a
hematogenous route, passing through
the walls of capillaries, which have
become permeable in the injury zone.
Endoneurial mast cells play a
pivotal role in this process,
proliferating markedly within the
first 2 weeks. They release
histamine and serotonin, which
enhance capillary permeability and
allow macrophage migration. During
the initial stages the endoneurial
tubes swell in response to the
trauma, but after the first 2 weeks
these tubes become smaller in
diameter. By 5-8 weeks, the
degenerative process is usually
complete, and the nerve fiber is
composed of Schwann cells within an
endoneurial sheath.
In
third-degree injuries, a more severe
local reaction to the trauma occurs.
These intrafascicular injuries
involve retraction of the severed
nerve fiber ends due to the elastic
endoneurium. Local vascular trauma
leads to hemorrhage and edema, which
results in a vigorous inflammatory
response. Fibroblasts proliferate,
and a dense fibrous scar results in
a fusiform swelling of the injured
segment. Interfascicular scar tissue
also develops so that the entire
nerve trunk, which is left in
continuity, is permanently enlarged.
Often, it is adherent to perineural
scar tissue as well.
Distal to the injured segment,
Wallerian degeneration follows a
sequence very similar to that
observed in second-degree injuries.
One important difference is that
intrafascicular injury impairs
axonal regeneration and, therefore,
the endoneurial tubes remain
denervated for prolonged periods.
Shrinkage of the endoneurial tubes
(diameter 2-4
µm)
reaches a maximum at approximately 4
months postinjury The endoneurial
sheath progressively thickens due to
collagen deposition along the outer
surface of the Schwann cell basement
membrane. If the endoneurial tube
does not receive a regenerating
axon, progressive fibrosis
ultimately obliterates the tube.
The stacked Schwann cell processes
comprising collapsed endoneurial
tubes have been labeled "bands of Büngner,"
Schwann cells appear to contribute
to the deposition of collagen, and
then revert to a more primitive form
indistinguishable from fibroblasts.
In addition to these degenerative
changes that occur distal to the
injured segment, retrograde changes
occur proximal to the injury site in
third-degree and in more severe
injuries.
In
fourth- and fifth-degree injuries,
local reaction to the severe trauma
is pronounced. Endoneurial tubes, as
well as fasciculi, are disrupted and
Schwann cells and axons are no
longer confined. The epineurium is
also damaged and reactive epineural
fibroblasts are present at the
severed nerve ends within 24 hours.
These are accompanied by
proliferating Schwann cells and
perineural and endoneurial
fibroblasts. Vigorous cellular
proliferation peaks within the first
week and continues for a prolonged
period. As in third-degree injuries,
capillary permeability increases,
probably as a result of mast cell
degranulation, and edema and
macrophage infiltration follow.
Each nerve end becomes a swollen
mass of disorganized Schwann cells,
capillaries, fibroblasts,
macrophages, and collagen fibers.
Regenerating axons reach the swollen
bulb of the proximal stump and
encounter formidable barriers to
further growth. Many axons form
whorls within the scar tissue, or
are turned back along the proximal
segment or out into surrounding
tissue. Some of the regenerating
axons may reach the distal stump. an
accomplishment which is dependent
upon multiple factors, including the
severity of the original injury,
the extent of scar formation, and
the delay before axons reach the
injury site. As in third-degree
injuries, endoneurial tubes left
unoccupied for prolonged periods
undergo progressive shrinkage and
fibrosis, ultimately becoming
completely obliterated by collagen
fibers.
Changes in neuronal cell bodies and
in nerve fibers proximal to the site
of injury depend upon the severity
of the injury as well as the
proximity of the injured segment to
the cell body. Schwann cells are
lost a few millimeters proximal to
the injured segment, and axons and
myelin are reduced in diameter. If
the cell body actually degenerates,
which occurs in severe trauma, the
entire proximal segment undergoes
Wallerian degeneration. The
Wallerian degeneration lags somewhat
behind this process in the distal
segment.
In
the presence of a surviving neuronal
cell body the axon is reduced in
diameter, particularly if functional
connections to appropriate end
organs are not re-established. Nerve
conduction velocity is accordingly
reduced, As regeneration proceeds,
the axonal diameters increase, but
may never reach normal levels, A
definite interdependence exists
between the cell body and the axon
in terms of recovery: the cell body
does not recover fully without the
re-establishment of functional
peripheral connections, and the
final axonal caliber depends to a
great extent upon the recovery of
the cell body.
The nerve cell body itself reacts in
a relatively predictable fashion
after axonal Injury. Within 6 hours
of the injury, the nucleus migrates
to the periphery of the cell and
Nissl granules break up and
disperse. This process is called
chromatolysis. Simultaneously, there
is a brisk proliferative response of
perineuronal glial cells, most
likely signaled by the process of
chromatolysis. Glial cell processes
extend to the affected neuron and
interrupt synaptic connections,
possibly to isolate the neuron for
its recovery phase. Some neurons go
on to degenerate and are
subsequently phagocytosed by
microglia.
More often, recovery begins within
2-3 weeks of the injury and
continues for up to several months.
The earliest signs of recovery are
the return of the nucleus to the
cell center and the reappearance of
compact Nissl granules. Subcellular
metabolic functions are altered
during the chromatolytic and
recovery phases, including an
increase in ribonucleic acid (RNA)
synthesis, a decrease in
neurotransmitter synthesis, and an
increase in production of proteins
and lipids needed for axonal
regeneration. Both fast and slow
components of axoplasmic transport
supply materials from the cell body
to the sites of axonal regeneration.
Regenerative Changes
In
nerve injuries of the first and
second degree (neurapraxia and
axonotmesis), restoration of
function is the rule. This is either
early through reversal of conduction
block, or late through axonal
regeneration. Functional recovery is
complete in these milder degrees of
injury. Both morphologic and
physiologic changes are fully
reversible.
In
the more severe nerve injuries in
which endoneurial tubes are
disrupted, regenerating axons are no
longer confined to their original
sheaths. These axons may meander
into surrounding tissue or into
inappropriate endoneurial tubes,
thus failing to reinnervate their
proper end organs. Neurologic
recovery is compromised, generally
to a degree proportional to the
severity of the injury.
Functional recovery after nerve
injury involves a complex series of
steps, each of which may delay or
impair the regenerative process. For
any degree of nerve injury, it is
useful initially to categorize these
regenerative steps anatomically on a
gross level. The sequence of
regeneration may be divided into
zones: (1) the neuronal cell body,
(2) the segment between the cell
body and the injury site, (3) the
injury site itself, (4) the distal
segment between the injury site and
the end organ, and (5) the end organ
itself. A delay in regeneration or
unsuccessful regeneration may be
attributed to pathologic changes
which impede normal reparative
processes at one or more of these
zones.
Neuronal Cell Body
Recovery of the neuronal cell body
is marked by a reversal of
chromatolysis and its associated
depression of protein synthesis.
Nucleoproteins reorganize into the
characteristic form of Nissl
granules. A complex and incompletely
understood interaction occurs
between the cell body and the
regenerating axon tip. Axoplasm,
which serves to regenerate the axon
tip, appears to arise in the axon
segment proximal to the injury site.
An intense increase in the rate of
protein synthesis in the cell
nucleus influences the rate of
advance and the final caliber of the
regenerating axon. The human
peripheral neuron's capacity to
initiate a regenerative response
appears to persist for at least 12
months after injury; and a robust
response can be elicited even after
repeated injuries.
Segment Between Cell Body and Injury
Site
The length of the segment between
the regenerating axon tip and the
injury site depends on the severity
of the original injury and the
consequent retrograde degeneration.
The first signs of axon regrowth in
this segment may be seen as early as
24 hours after injury, they may be
delayed for weeks in more severe
degrees of injury. The rate of
axonal regrowth is determined by
changes within the cell body, the
activity of the specialized growth
cone at the tip of each axon sprout,
and the resistance of the injured
tissue between cell body and end
organ.
There may be multiple axon sprouts
within each endoneurial sheath, even
in milder injuries, which do not
involve destruction of the sheath
itself. The fate of these multiple
sprouts is not clear even in
experimental paradigms. The timing
of degenerative and regenerative
processes is such that there must be
a significant overlap between these
in certain segments (Figure 2). For
example, in milder injuries in which
there is no significant delay in
regeneration across the injury site,
the advancing axon tip must
encounter the debris of Wallerian
degeneration in the distal segment.
This debris does not appear to
impose a barrier to regeneration.
However, in very proximal injuries
in which there is a considerable
delay before the advancing axon tip
reaches the distal segment, the
empty endoneurial tubes distally
have decreased in diameter. This
factor may be responsible, in part,
for a terminal slowing in axonal
regrowth. Surgical intervention that
interrupts entering nutrient
arteries does not appear to impair
axonal regeneration, provided that
longitudinal arteries within the
nerve itself are not disrupted.
Injury Site
In
severe nerve injuries that disrupt
the endoneurial tubes, nerve
fascicles, or trunks, formidable
obstacles face the regenerating
axons that reach the injury site.
There may be a gap between the
disrupted nerve ends, allowing
regenerating axon sprouts to wander
into surrounding tissue. Scarring is
inevitably present at the site of
severe injury; the extent depends
upon multiple factors, including the
timing of arrival of the
regenerating sprouts after injury.
It
has been well documented that
regenerating axons may at times
successfully traverse long gaps
spontaneously, despite the presence
of substantial scar tissue. However,
there is no question that an
appropriate surgical repair can
eliminate the gap and reduce the
amount of intervening scar tissue.
This procedure provides no guarantee
of proper fascicle orientation, of
course, and regenerating axons may
grow into functionally inappropriate
endoneurial tubes or even may fail
to re-enter an endoneurial tube.
Either circumstance results in
wasted axons.
Previously nonmyelinated axons may
regenerate into endoneurial sheaths
which formerly contained myelinated
axons (and vice versa). This
regeneration will not be wasteful.
The resistance that an axon meets at
the injury site results in the
formation of multiple smaller axon
sprouts. These daughter axons do not
all find their way into the distal
segment. No specific neurotropism is
known to enhance the growth of a
regenerating axon into its original
endoneurial tube, but some form of
neurotropic influence has been
demonstrated in experimental
paradigms. Scarring within the
bridging tissue impedes regeneration
and misdirects axon sprouts into
functionally unrelated endoneurial
tubes. Residual scar tissue also
interferes with the maturational
processes of axons that do negotiate
the injury site.
Segment
Between Injury and End Organ
Axons that successfully enter
endoneurial tubes in the segment
distal to the injury site stand a
good chance of reaching the end
organ, given reasonable growth
conditions. The distal regeneration
rate is slower if the endoneurial
tubes have been disrupted. The
specialized growth cone at the tip
of each axon sprout contains
multiple filopodia, which adhere to
the basal lamina of the Schwann
cell. Several small axon sprouts may
enter the same endoneurial tube.
Hence, a regenerated nerve fiber may
contain more axons than the original
nerve.
If
a functionally unrelated end organ
is reached, further development of
the axon and remyelination do not
occur. Similarly, axonal development
and maturation are aborted if the
end organ, due to prolonged
denervation, has undergone
degenerative changes that do not
allow the establishment of
functional connections. If the entry
of regenerating axons into the
distal segment is delayed more than
approximately 4 months, the axons
are entering endoneurial tubes of
small diameter, generally 3
µm
or less. This
shrinkage does not appear to impede
regeneration or to impair functional
recovery, most likely due to the
elastic properties of the
endoneurium.
The return of function does not
require absolutely faithful recovery
of nerve fiber architecture. The
effects of prolonged denervation,
which do appear to impair functional
recovery, are at the level of the
injury site-i.e. preventing the
regenerating axons from entering
appropriate endoneurial tubes-or at
the end organ.
End Organs
End organs undergo characteristic
histologic changes with nerve
degeneration and subsequent
reinnervation. Muscle fibers atrophy
quite rapidly (70% average reduction
of cross-sectional area by 2 months)
and cell nuclei assume a central
rather than the normal peripheral
position. The synaptic folds of
motor end plates are preserved for
at least a year after denervation.
Tremendous proliferation of
fibroblasts also characterizes the
histologic picture of denervation.
New collagen is deposited in both
the endomysium and perimysium. In
general, muscle fibers are not
replaced by connective tissue, but
rather atrophied fibers are
separated by thickened connective
tissue, so that the overall internal
pattern of muscle architecture is
preserved. Occasional dropout of
muscle fibers does occur. This is a
relatively late phenomenon,
generally observed between 6- 12
months after denervation.
Regenerating axonal sprouts follow
the original Schwann cells to the
denervated motor end plates to
reform neuromuscular junctions.
Collateral sprouting also occurs,
resulting in groups of reinnervated
muscle fibers, all of the same fast
or slow types. This is a
characteristic finding in
reinnervated muscle, contrasting
sharply with the random pattern
observed in normal muscle.
Unfortunately, incomplete motor
recovery is a common occurrence
after moderate-to-severe nerve
injuries. This is due to a number of
factors, within the muscle itself
and in the regenerating nerve.
Intramuscular fibrosis may limit the
efficiency of the contraction
produced by a nerve impulse.
Appropriate physical therapy can be
an important intervention that
maintains the denervated muscles in
an optimal condition to receive the
regenerating axon terminals.
The role of electrical stimulation
of denervated muscle or of
regenerating nerve remains
controversial. Motor recovery is
obviously impaired if a significant
number of axons do not successfully
reform functional connections with
the muscle. Even if the numbers are
adequate, erroneous
cross-reinnervation may produce a
suboptimal functional result: an
originally "fast" muscle may be
reinnervated by axons previously
innervating a "slow" muscle, and the
result may be a mixed form with
inefficient contraction.
Concomitant sensory deficits,
particularly in proprioception,
further impede functional motor
recovery. A variety of explanations
have been proposed for the generally
poor recovery of intrinsic hand
muscles after a severe, proximal
upper extremity nerve injury with or
without nerve repair. One of the
explanations most commonly preferred
is a loss of motor end plates in the
denervated muscles due to the long
delay before reinnervation occurs.
While this factor may well play a
role, Sunderland seriously
questioned its overall significance
and cited instead a number of
primarily neurogenic factors for the
disappointing recovery of hand
function.
Denervated sensory receptors survive
and may make useful functional
recoveries after 1 year and possibly
after many years. In first- and
second-degree injuries, return of
sensation is complete in its
original pattern, even after 6-12
months of denervation. This is due
to faithful reinnervation of sensory
receptors by their original axons.
After more severe injuries and nerve
repair, sensory recovery is never
complete. This is undoubtedly due to
a combination of factors, including
failure of sensory axons to reach
the skin, cross-innervation (an axon
originally from one type of receptor
making connections with a different
type of receptor), and possibly
degeneration of sensory receptors.
Some controversy exists over the
fate of denervated encapsulated
sensory receptors, the Pacinian and
Meissner's corpuscles (rapidly
adapting receptors mediating light
touch and vibration), and the Merkel
cells (slowly adapting receptors
mediating constant touch and
pressure). It is believed that these
specialized receptors survive in an
atrophied state for prolonged
periods of time, awaiting the
arrival of an appropriate nerve
terminal. The survival period has
not been clearly established,
however, and there is some evidence
to suggest that the protective
sensation, which recovers years
after denervation, is mediated by
less elaborate sensory receptors.
The rate of axon regeneration has
been assumed to be constant, and in
clinical situations is generally
estimated to be 1 mm per day.
However, reported rates of
regeneration vary over a broad
range, from 0.5-9.0 mm per day. This
variability is due to several
factors: (1) the rate of axon growth
decreases with increasing distance
from the cell body to the advancing
axon tip; (2) measurements of axonal
regeneration were made in different
species after different methods of
nerve injury; and (3) the techniques
for measuring regeneration were
different. Moreover, the rate of
regeneration can vary, depending on
the nature and severity of the nerve
injury, the duration of denervation,
and the condition of the peripheral
tissues. Regeneration after surgical
nerve repair is slower than
uncomplicated regeneration, most
likely reflecting the severity of
the original injury.
In
addition to axonal regeneration, a
process of maturation precedes
functional recovery. Morphologic
changes of maturation proceed along
the regenerating axon at a slower
rate than axon regrowth and continue
for a protracted period-as long as 1
year. Remyelination takes place in a
manner similar to that for
developing nerve fibers, involving
alignment of Schwann cells and
encircling of the axon to form a
multilamellated sheath. This process
begins within 2 weeks of the onset
of axonal regeneration and results
in myelinated axons quite similar to
the originals except for shortened
internodes. Axonal diameter
increases progressively until normal
dimensions are reached. This
enlargement is dependent upon the
establishment of functional
connections between the axon tip and
the appropriate end organ.
Electrophysiology of Nerve
Regeneration
Electromyography (EMG) is a useful
laboratory adjunct in the evaluation
of patients with peripheral nerve
injuries. The appropriate
application of EMG, it should be
emphasized, is performed as a
supportive technique rather than as
a replacement for clinical
diagnosis. The technique is very
useful in the evaluation of patients
with clinically complete lesions who
may have a few surviving motor
fibers, as it can demonstrate nerve
continuity. EMG also provides the
earliest and most sensitive
indicator of reinnervation of
denervated muscles.
Within 10-18 days of denervation,
fibrillations occur in muscle fibers
in the form of fine, rapid,
irregular rippling contractions that
cannot be detected clinically
through the skin. On EMG these are
represented by spontaneous
fibrillation potentials (Figure 2),
which are lowamplitude (100-300
µV)
mono- or biphasic spikes. The spikes
are largely negative in polarity
following an initial positive
deflection, and of short duration
(1-2 msec). Fibrillation potentials
indicate a nerve injury severe
enough to produce Wallerian
degeneration-Sunderland second
through fifth degrees.
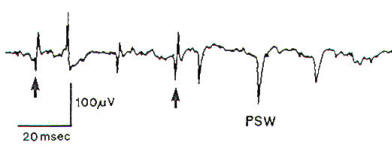 |
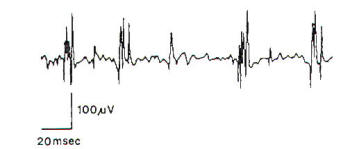 |
Fig-2:
EMG - Fibrillation
potentials (arrows) and
positive sharp waves
(PSW), indicating acute
denervation. |
Fig-3:
EMG showing long
duration, small
amplitude polyphasic
motor unit
potentials, indicating
the regeneration. |
Spontaneous positive sharp waves may
also be observed (Figure 2). These
are characterized by steeply rising
positive (downward) deflections of
large amplitude (50-4000
µV),
followed by a slow descent lasting
up to 200 msec. Increased
insertional activity is observed in
all forms of denervation. In partial
denervation, the number of motor
units with voluntary contraction is
reduced. This results in an
incomplete interference pattern.
Reinnervation is characterized on
EMG by a reduction and, ultimately,
a disappearance of fibrillation
potentials as well as the appearance
of reinnervation potentials (Figure
3). The latter are low-amplitude,
very long duration polyphasic
potentials, which must be
differentiated from the polyphasic
potentials of normal duration
occasionally observed in normal
muscle. (Normal muscle typically has
< 10% polyphasic motor unit
potentials.) These reinnervation
potentials are reliable guides to
the onset of muscle fiber
reinnervation, but are not
necessarily predictive of functional
voluntary contraction.
For useful return of function, there
must be reinnervation by
regenerating axons in sufficient
numbers. The EMG cannot quantitate
this. Kline's technique of
intraoperative nerve action
potential (NAP) recording provides
the most reliable guide to
clinically useful recovery after
nerve injury. He found that more
than 90% of patients in whom NAP can
be recorded across a
lesion-in-continuity make an
acceptable functional recovery
without resection of the lesion and
nerve repair.
Nerve conduction velocity (NCV)
studies also provide information
relevant to nerve injury and
regeneration. When performed 7-10
days after nerve injury, NCV studies
may distinguish between conduction
block (neurapraxia) and axonal loss.
The preservation of the distally
invoked compound muscle action
potential (which may be seen even in
complete paralysis), indicates
proximal conduction block rather
than Wallerian degeneration. The
latter results in loss of amplitude
proportionate to the number of axons
lost. The time course of axon
regeneration and maturation may be
followed, as well as assessment of
the outcome of regeneration.
However, NCV studies do not provide
any prognostic information regarding
the return of useful motor function.
Following uncomplicated regeneration
without surgical nerve repair,
conduction velocity slowly returns
toward normal over 6-15 months.
After surgical repair, the
conduction velocity never returns to
normal and improves even more
slowly.
Future Directions
Basic neurobiology research has
advanced our understanding of the
fundamental mechanisms of nerve
growth and regeneration.
In
practical terms, this has not yet
translated into a great deal of
useful information for the
peripheral nerve surgeon. Clinical
and laboratory studies of practical
utility have dealt with techniques
and timing of nerve repair, as well
as the appropriate selection of
patients for surgery. Future studies
will likely involve the development
of methods for enhancing axonal
regeneration, including transport
systems, growth factors, specific
neurotropic factors, and possibly
the use of nerve allografts or
synthetic bioabsorbable conduits.
Axonal transport systems play an
important role in the normal
maintenance of nerve processes and
in regenerative efforts-conveying
structural proteins, enzymes, and
organelles to and from the advancing
axon tip. Adjustments in fast and
slow transport systems are to be
expected during regeneration, and
have been measured in various
experimental paradigms. The
literature to date contains
conflicting reports regarding the
nature of these changes . A greater
understanding of the regulatory
mechanisms controlling these
transport systems may allow
manipulation of axonal transport to
effect more efficient regeneration.
New techniques are being developed
to study transport mechanisms.
Neurite growth-promoting factors
have been revived as subjects of
intense investigation.
Levi-Montalcini and colleagues
initiated this field with pioneering
studies of nerve growth factor (NGF)
in the 1950. This polypeptide
induces sprouting of sympathetic and
sensory neurons in tissue culture;
it plays an important role in vivo
in the regulation of growth and the
development and maintenance of these
neurons in sympathetic and dorsal
root ganglia. The nature of its role
in axonal regeneration after nerve
injury and repair is poorly
understood. It is presumably
produced by Schwann cells (among
others), and is transported in a
retrograde manner to neuronal cell
bodies. In situ hybridization
studies of NGF receptor mRNA suggest
a role for NGF in motor neuron
regeneration.
Other neurite growth-promoting
factors have captured the spotlight
in nerve regeneration research.
Acidic fibroblast growth factor
(aFGF) is the first highly purified
protein since NGF that has been
shown to enhance nerve regeneration
in vivo. A collagen-aFGF mixture
inside a polyethylene guide tube
increases axonal growth across a gap
in a transected rat sciatic nerve.
Whether this is a direct effect on
neurons, on angiogenesis, or on
non-neuronal cells has not been
determined. Components of the
extracellular matrix and basement
membranes have been examined for
their potential neurotropic effects.
The glycoprotein laminin, the major
noncollagenous protein of basement
membranes, has received considerable
attention because of its ability to
enhance neurite outgrowth both in
vitro and in vivo. A novel homologue
of laminin, s-laminin, has been
identified in association with the
basal lamina of the synaptic cleft.
This glycoprotein may be
responsible, in part, for the
striking topographic specificity of
synapse formation demonstrated by
regenerating motor axons on
denervated muscle fibers. P30, a
heparin-binding protein with cell
adhesive properties, promotes
neurite outgrowth in developing rat
central nervous system and appears
to play a role in interactions
between neurons and Schwann cells in
regenerating peripheral nerves.
Gangliosides have also been proposed
as neurite growth promoters in both
in vitro and in vivo studies.
Electromagnetic field and direct
current stimulation of regenerating
axons has received a great deal of
investigative attention, analogous
to the orthopedic studies of these
modalities in bone healing. While
the latter studies demonstrated a
beneficial effect on fracture
healing related to increased
collagen deposition, the nerve
regeneration studies have not been
conclusive. A variety of biological
and synthetic nerve conduits have
been proposed as replacements for
nerve autografts in nerve repair:
these include prepared skeletal
muscle, collagen membrane, arterial
and nerve allografts, polyglycolic
acid, and Silastic. Proposed
replacements for traditional suture
repair techniques include fibrin
glue and laser welding. Although
none of these techniques has
demonstrated convincing advantages
in the clinical setting over the
"gold standard" of the nerve
autograft and careful microsurgical
suture repair, there are promising
theoretical arguments for pursuing
them. |